Search Your Topic
Electron Transport Chain -strictly aerobic (a brief review)
Introduction
Most of the energy liberated during the oxidation of carbohydrates, fatty acids, and amino acids are made available within mitochondria as reducing equivalents (—H or electrons). The NADH and FADH2 formed in glycolysis, fatty acid oxidation, and the citric acid cycle are energy-rich molecules because each contains a pair of electrons having a high transfer potential. The enzymes of the citric acid cycle and β-oxidation are contained in mitochondria, together with the respiratory chain, which collects and transports reducing equivalents, directing them to their final reaction with oxygen to form water. Hence the electron transport chain is operative only in the presence of oxygen, it is strictly aerobic.
Oxidative phosphorylation
The reduction of molecular oxygen to water yields a large amount of free energy that can be used to generate ATP. Oxidative phosphorylation is the process in which ATP is formed as a result of the transfer of electrons from NADH or FADH2 to O2 by a series of electron carriers. This process, which takes place in mitochondria, is the major source of ATP in aerobic organisms.
Components of Electron Transport Chain
Electrons flow through the respiratory chain through a redox span of 1.1V from NAD+/NADH to O2/2H2O, passing through three large protein complexes; NADH-Q oxidoreductase (Complex I), where electrons are transferred from NADH to coenzyme Q (Q) (also called Ubiquinone); Q-cytochrome c oxidoreductase (Complex III), which passes the electrons on to cytochrome c; and cytochrome c oxidase(Complex IV), which completes the chain, passing the electrons to O2and causing it to be reduced to H2O (Figure-1). Some substrates with more positive redox potentials than NAD+/NADH (eg, succinate) pass electrons to Q via a fourth complex, succinate Q reductase (Complex II), rather than Complex I. The four complexes are embedded in the inner mitochondrial membrane, but Q and cytochrome c are mobile. Q diffuses rapidly within the membrane, while cytochrome c is a soluble protein.
Flavoproteins
Flavoproteins (are important components of Complexes I and II. The oxidized flavin nucleotide (FMN or FAD) can be reduced in reactions involving the transfer of two electrons (to form FMNH2 or FADH2), but they can also accept one electron to form the semiquinone. Iron-sulfur proteins (non-heme iron proteins, Fe-S) are found in Complexes I, II, and III. These may contain one, two, or four Fe atoms linked to inorganic sulfur atoms and/or via cysteine-SH groups to the protein. The Fe-S takes part in single electron transfer reactions in which one Fe atom undergoes oxidoreduction between Fe2+ and Fe3+.
Electron flow in ETC
NADH-Q oxidoreductase or Complex I is a large L-shaped multi-subunit protein that catalyzes electron transfer from NADH to Q, coupled with the transfer of four H+across the membrane: Electrons are transferred from NADH to FMN initially, then to a series of Fe-S centers, and finally to Q (Figure-1). In Complex II(succinate -Q reductase), FADH2 is formed during the conversion of succinate to fumarate in the citric acid cycle and electrons are then passed via several Fe-S centers to Q.
Coenzyme Q or Ubiquinone (Ubi)
Ubi accepts electrons and transports them to Complex III. Ubi is capable of moving in the membrane between Complex I and III, picking up electrons at Complex I and dropping them off at Complex III. As is the case with Complex I, Complex III contains a series of proteins that transport electrons. As the electrons are moved from carrier to carrier within Complex III, the energy that is released moves hydrogen ions out of the mitochondrion. Thus, Complex III also serves as a hydrogen ion pump. Once again, there must be a mechanism by which electrons are removed from Complex III. This is done by a small peripheral membrane protein known as cytochrome c. Cytochrome c transports the electrons from Complex III to Complex IV.
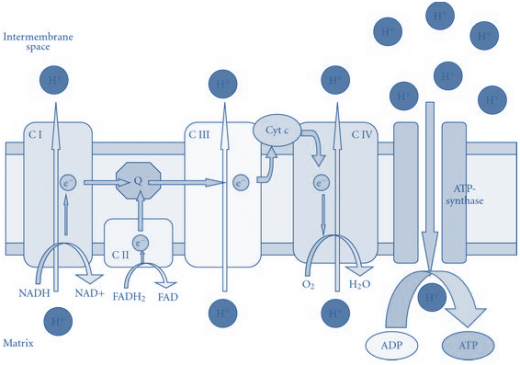

Figure-1- showing the components of the electron transport chain
Reduced cytochrome c is oxidized by Complex IV (cytochrome c oxidase), with the concomitant reduction of O2 to two molecules of water:
This transfer of four electrons from cytochrome c to O2 involves two heme groups, a and a3, and Cu. Electrons are passed initially to a Cu center (CuA), which contains 2 Cu atoms linked to two protein cysteine-SH groups (resembling a Fe-S), then in sequence to heme a, heme a3, a second Cu center, CuB, which is linked to heme a3, and finally to O2.
By the time the electrons reach the final electron acceptor in Complex IV, they have very little energy left and need to be removed entirely from the system(otherwise the entire system will back up and be halted). Complex IV finally gives its electrons to molecular oxygen (O2), the final or terminal electron acceptor. The reduction of oxygen (remember, accepting electrons is a reduction reaction) results in the formation of a water molecule from the oxygen. For every pair of electrons donated to the ETC by an NADH, ½ O2(or one O atom) is reduced to form one water molecule. Two NADH’s would result in the use of 1 (½ + ½) O2 molecule, and so on. If there was no oxygen to take the electrons from Complex IV, the electrons would accumulate up the electron transport chain very quickly and NADH would no longer be able to donate electrons to the ETC (because the ETC would be filled with electrons). This would cause an accumulation of NADH and a shortage of NAD+(remember, NAD+ is regenerated when NADH donates its electrons to Complex I). Without NAD+, there would be no electron acceptors for the Krebs Cycle and all reactions that require NAD+ would stop. This would cause the entire Krebs Cycle to stop. Thus the Krebs Cycle would not run if there were no oxygen present, thus it is considered aerobic!
Proton Motive Force
The flow of electrons from NADH or FADH2 to O2 through protein complexes located in the mitochondrial inner membrane leads to the pumping of protons out of the mitochondrial matrix(figure1). The resulting uneven distribution of protons generates a pH gradient and a transmembrane electrical potential that creates a proton-motive force (Figure -2). ATP is synthesized when protons flow back to the mitochondrial matrix through an enzyme complex (Figure -1). Thus, the oxidation of fuels and the phosphorylation of ADP are coupled by a proton gradient across the inner mitochondrial membrane.
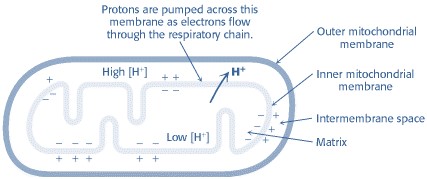

Figure-2-showing the electrochemical gradient created by the pumping of protons across the inner mitochondrial membrane
In other words, the electron-motive force is converted into a proton-motive force and, finally, the proton-motive force is converted into phosphoryl transfer potential.
Like NADH, FADH2 contains electrons (and energy) from oxidation reactions. Remember that FADH2, however, contains less energy than does NADH. Because of this, FADH2 is unable to donate its electrons to Complex I of the ETC. Instead, FADH2 donates its electrons to Complex II. Complex II eventually passes the electrons on to Complex III via Ubiquinone. From then on, the electrons follow the same pathway as do those from NADH. Complex II, it turns out, does not move protons as the electrons are transported. This means that every NADH that donates electrons to the ETC will cause more hydrogen ions to be transferred than does each FADH2.
P: O ratio
There is a net direct capture of two high-energy phosphate groups in the glycolytic reactions. Two more high-energy phosphates per mole of glucose are captured in the citric acid cycle during the conversion of succinyl CoA to succinate. All of this phosphorylation occurs at the substrate level. When substrates are oxidized via Complexes I, III, and IV in the respiratory chain (ie, via NADH), 3 mol of ATP are formed per half mol of O2consumed; ie, the P:O ratio = 3. On the other hand, when a substrate (e.g.succinate) via FADH2 is oxidized through Complexes II, III, and IV, only 2 mol of ATP are formed; ie, P:O =2. These reactions are known as oxidative phosphorylation at the respiratory chain level. Taking these values into account, it can be estimated that nearly 90% of the high energy phosphates produced from the complete oxidation of 1 mol glucose is obtained via oxidative phosphorylation coupled to the respiratory chain
Inhibition of Oxidative phosphorylation
Oxidative phosphorylation is susceptible to inhibition at all stages of the process.
1) Site-specific inhibitors –Specific inhibitors of electron transport, for example, rotenone and amobarbital block electron transfer in NADH-Q oxidoreductase and thereby prevent the utilization of NADH as a substrate. In contrast, electron flow resulting from the oxidation of succinate is unimpaired, because these electrons enter through QH2, beyond the block. BAL(British Anti Lewisite), Antimycin A interfere with electron flow from cytochrome b in Q-cytochrome c oxidoreductase. Furthermore, electron flow in cytochrome c oxidase can be blocked by cyanide (CN-), azide (N3 -), and carbon monoxide (CO). Cyanide and azide react with the ferric form of heme a 3, whereas carbon monoxide inhibits the ferrous form. Inhibition of the electron-transport chain also inhibits ATP synthesis because the proton-motive force can no longer be generated. Malonate is a competitive inhibitor of Complex II
2) ATP synthase- also can be inhibited. Oligomycin and dicyclohexyl carbodiimide(DCCD) prevent the influx of protons through ATP synthase. If actively respiring mitochondria are exposed to an inhibitor of ATP synthase, the electron transport chain ceases to operate. Indeed, this observation clearly illustrates that electron transport and ATP synthesis are normally tightly coupled.
3)Inhibition of ATP-ADP translocase-ATP-ADP translocase is specifically inhibited by very low concentrations of Atractyloside(a plant glycoside) or bongregate (an antibiotic from a mold). The unavailability of ADP also inhibits the process of ATP formation. This is because oxidation and phosphorylation are tightly coupled; ie, oxidation cannot proceed via the respiratory chain without concomitant phosphorylation of ADP.
4)Uncouplers of Oxidative phosphorylation- This tight coupling of electron transport and phosphorylation in mitochondria can be disrupted(uncoupled) by 2,4- dinitrophenol, 2,4 dinitrocresol and certain other acidic aromatic compounds. These substances carry protons across the inner mitochondrial membrane. In the presence of these uncouplers, electron transport from NADH to O2 proceeds in a normal fashion, but ATP is not formed by mitochondrial ATP synthase because of the proton-motive force across the inner mitochondrial membrane is dissipated. This loss of respiratory control leads to increased oxygen consumption and oxidation of NADH. Indeed, in the accidental ingestion of uncouplers, large amounts of metabolic fuels are consumed, but no energy is stored as ATP. Rather, energy is released as heat.
The regulated uncoupling of oxidative phosphorylation is a biologically useful means of generating heat. The uncoupling of oxidative phosphorylation is a means of generating heat to maintain body temperature in hibernating animals, in some newborn animals (including human beings), and in mammals adapted to cold. Brown adipose tissue, which is very rich in mitochondria (often referred to as brown fat mitochondria), is specialized for this process of nonshivering thermogenesis. The inner mitochondrial membrane of these mitochondria contains a large amount of uncoupling protein (UCP), here UCP-1, or thermogenin, a dimer of 33-kd subunits that resembles ATP-ADP translocase. UCP-1 forms a pathway for the flow of protons from the cytosol to the matrix. In essence, UCP-1 generates heat by short-circuiting the mitochondrial proton battery.
Thus, the manner in which biologic oxidative processes allow the free energy resulting from the oxidation of foodstuffs to become available and to be captured is stepwise, efficient, and controlled—rather than explosive, inefficient, and uncontrolled, as in many non-biologic processes. The remaining free energy that is not captured as high-energy phosphate is liberated as heat. This need not be considered “wasted,” since it ensures that the respiratory system as a whole is sufficiently exergonic, allowing continuous unidirectional flow and constant provision of ATP. It also contributes to the maintenance of body temperature.